US Electron Ion Collider to be Built at Brookhaven National Laboratory
By Abhay Deshpande, Professor, Stony Brook University; Director, EIC Science, Brookhaven National Laboratory
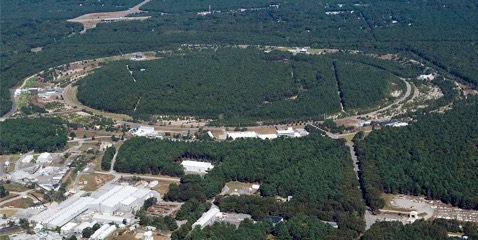
Recent news and excitement
On January 9, 2020, the US Department of Energy (DOE) announced the selection of Brookhaven National Laboratory (BNL) as the site of its future Electron Ion Collider (EIC). The EIC will be built over the next ten years with an estimated cost between $1.6 and $2.6 billion. With this announcement ended a two-decade long phase of making the scientific case for the collider within the broader science community: The Nuclear Science Advisory Committee’s Long Range Planning discussions in 2002, 2007 and 2015 [1], an independent assessment of the EIC science by the National Academy of Science, Engineering & Medicine (NAS) in October 2018 [2] followed by official start of the project (Critical Decision 0) within the Office of Science in December 2019, and finally, the site selection in January 2020. EIC realization begins with BNL and Jefferson Lab working closely together with the DOE and the potential EIC Users Group to realize its science in the 2030’s.
The visible universe is made of atoms of various naturally occurring elements. At the heart of the atom are nuclei, made of protons and neutrons (collectively called hadrons). Electrons fly around these nuclei in well-defined and well understood orbits. The interactions amongst the electrons and the nuclei are mediated by photons, the carriers of the electroweak force – an extremely well understood force of nature. Photons themselves are chargeless. They can only interact with electrically charged particles, such as protons, neutrons and other electrons, but can’t interact with other photons.
Inside the hadrons is the realm of strong interactions, mediated by the force carrier called the “gluon”. Gluons are also massless, and electrically neutral but carry a novel “color” charge, which enables them to interact not only with the colored quarks and antiquarks but also amongst themselves. This ability of gluon self-interaction leads to profoundly important consequences. A color charge in isolation can initiate gluon radiation, and due to its ability to self-interact, lead to an uncontained growth in gluon number resulting in a
“thundercloud” [3]. Nature controls this by confining color inside hadrons with finite energy, tames this growth and prevents the thundercloud. Such color interactions lead to the incredible richness and complexity of the strong interactions.
Quantum Chromodynamics (QCD), the theory of strong interactions within the Standard Model of Physics, is unquestionably the right theory that describes the interactions amongst partons (quarks and gluons) inside the hadrons. The 2004 Nobel Prize in Physics was awarded to David Gross, H. David Politzer and Frank Wilczek “for their discovery of asymptotic freedom in the theory of strong interactions” within the framework of QCD, and yet many fundamental and compelling questions related to gluon’s role in QCD remain unanswered. Without gluons there would be no hadrons, no atomic nuclei, no galaxies—no visible matter. The Higgs Boson which is often credited for imparting “mass” to the universe, actually provides less than 1% of the mass of the visible universe. The rest comes from the partonic interactions, including in large part driven by gluons. How this happens exactly is not understood, but astonishingly, the massless gluons and almost massless quarks, through their interactions make up the entire visible matter and its mass in the Universe. Precision study of the gluon and their interaction is hence called “the next QCD frontier” [4].
Using the precision enabled by the well understood electroweak force the EIC will study the quark-gluon structure and their dynamics in the hadrons and in nuclei with unprecedented precision. EIC is the only collider ever designed to collide polarized electrons with polarized protons, deuterons, and a few light nuclei and with all stable nuclei over a broad range of center of mass energies. This makes the EIC one of the most technically demanding accelerator facilities in the world. In the National Academy’s consensus report [2], it was hence noted that the EIC would not only help maintain U.S. leadership in nuclear physics, but also in the accelerator science and technology.
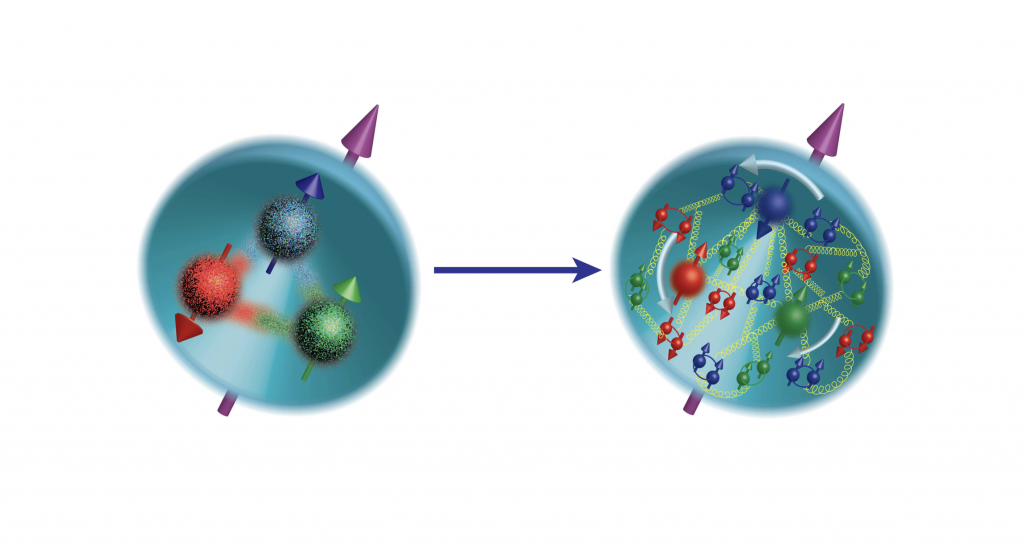
The Science Opportunities at the Electron Ion Collider
Origin of Mass and Spin
Most of the mass of the visible universe comes from the protons and neutrons in the nuclei of the atoms. Electrons themselves contribute very little. Protons and neutrons are made of almost massless quarks, antiquarks and the massless gluons. Together they contribute to barely 2% of the protons mass. Where does the rest of the mass come from? It is expected that the motion of quarks and the energy of the gluons resulting from their interactions must contribute in the total relativistic energy of the system contributing to the remaining 98% of the proton’s mass, see Figure 2 (Right). Exactly how this happens remains a mystery.
Nucleon’s spin is similarly an enigma. Quarks are fermions (spin ½ particles) and gluons are bosons (spin 1). Their spin alignment was until recently expected to contribute and explain the entire spin of a nucleon. However, experiments at CERN, SLAC and DESY in the late 1980’s, and the 1990’s revealed that in fact, quarks and anti-quarks together only contribute about 25% of the nucleon spin. In 2000’s the PHENIX and STAR experiments at RHIC with polarized protons have revealed that the gluon-spin contribution to the nucleon’s spin also seems to be about 25%. Where does the remaining 50% spin of the nucleon come from? Experimental evidence of transverse motion of quarks and anti-quarks inside the nucleon leads to the picture of quarks and anti-quarks having orbital motion) in the sea of gluons. Those orbital momenta collectively should contribute to the nucleon’s total angular momentum explaining the total the nucleon spin (Figure 2: Right). The same relativistic motion of quarks and gluons naturally explain origin of the mass of the nucleon. A direct measurement and observation of orbital motion of quarks and gluons is needed. A detailed and systematic study of direct measurement of this orbital motion by studying the spatial and momentum correlations of quarks and gluons is one of the most important goals of the EIC [4].
Novel Cold Dense Gluonic Matter
Nuclei are accurately modeled as collection colorless protons and neutrons interacting with each other through long range forces mediated by the exchange of pions. There is another regime predicted in QCD at high energy where quarks become static sources of gluon fields reaching extreme high density. The EIC would be able to experimentally investigate this high energy regime of QCD employing electron scattering off high energy protons and heavy nuclei which are known to have very large number of gluons. The HERA collider (the high energy e-p collider that operated at DESY 1992-2006) found that the number of gluons grows exponentially inside the protons at high energy. In nuclei, a significantly higher gluon densities are expected than in protons at the comparable energies. The gluons in the nuclei are expected to overlap in the transverse position inside the nuclei and reach a highly dense quantum state of “cold dense gluonic matter”. This novel state, often called the “Color Glass Condensate (CGC)” could be the QCD analogue of the Bose-Einstein Condensate of cold atoms studied in atomic physics. To experimentally measure this effect, we would use nuclei at the highest possible energy available at the EIC, and shine the virtual light originating from high energy electron as shown in Figure 3.
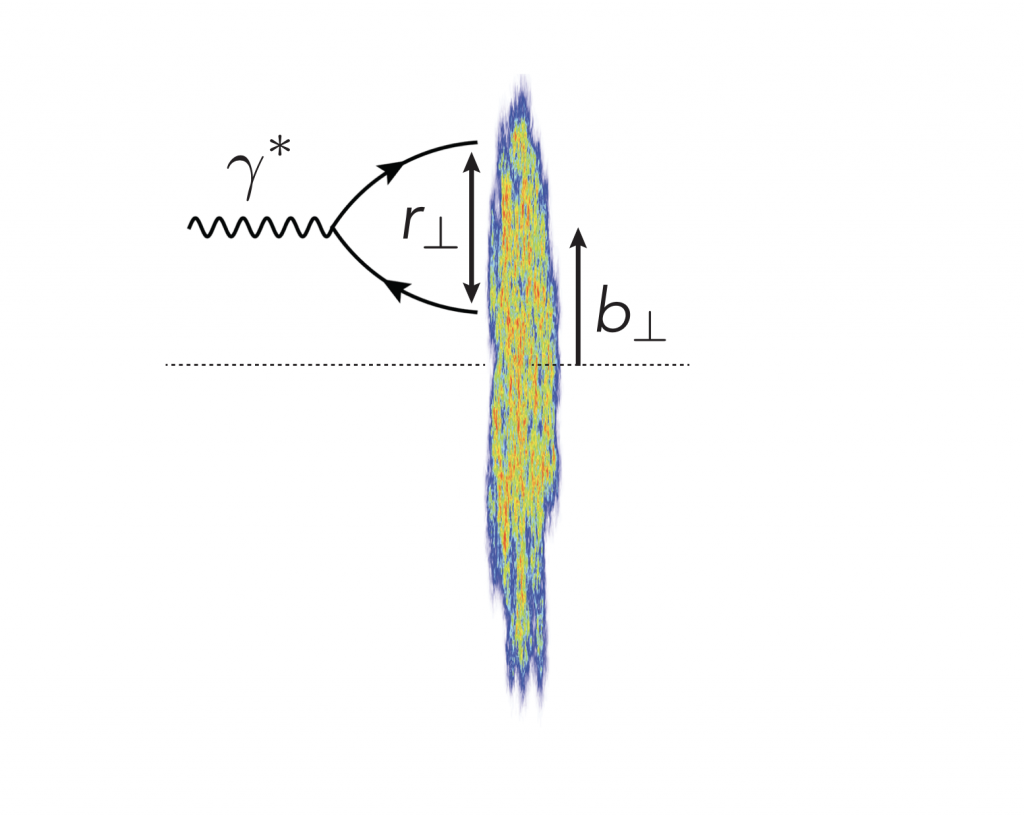
Figure 3 shows a schematic of a quark-antiquark dipole in DIS colliding with a high energy nucleus enabling gluon exchange. A process identical to “diffraction of mono-chromatic light off an opaque object” is expected to occur due to the highly dense gluonic object formed in nuclei at high energies, resulting in a “diffraction pattern” in particle production. A large fraction of e-A scattering will be channeled into such diffractive scattering should such “wall of glue” exist. Experiments at HERA saw hints of such effect. The high density of gluons possible using the nuclear beams at the highest energy, the EIC will decisively produce robust signatures of CGC if it exists.
Color Interactions in Nuclei, Hadronization
Figure 4 shows a schematic of the parton moving through the nucleus after being kicked by the virtual photon emitted from the electron. The parton must neutralize its color by picking up appropriate partners as it passes through the nucleus. This color neutralization process results in the formation of color neutral mesons (called “hadronization”) detected in the detector. At high enough energies, a “jet” of color charged particles (instead of a single charge) could be traversing through the nucleus. The EIC and an appropriately designed detector would enable detailed studies of hadronization by exquisite control over energy and the amount of the nuclear medium by controlling the size of the nucleus.
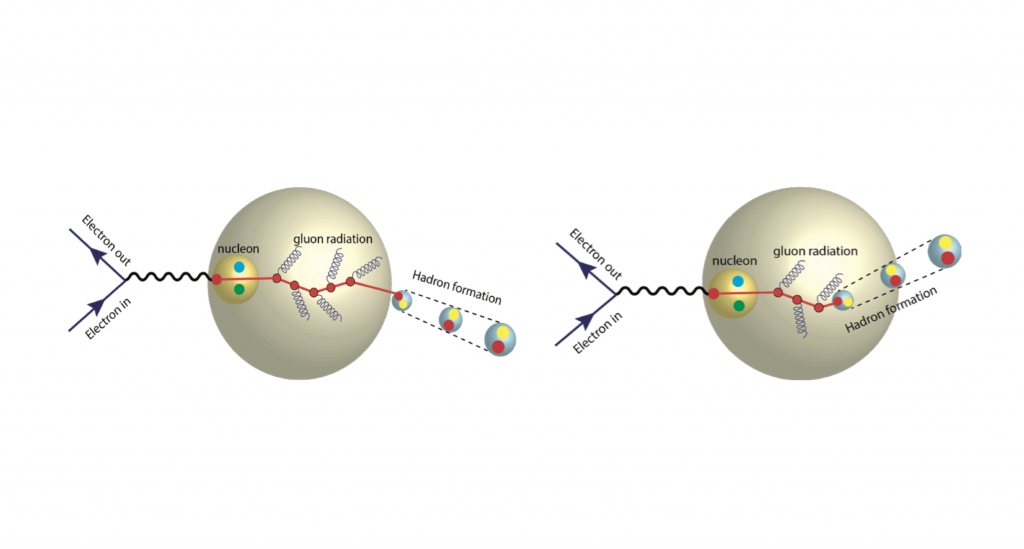
The Electron Ion Collider at Brookhaven National Laboratory
The layout of the EIC at BNL is shown in Figure 5. The EIC will require addition of a 5-18 GeV electron storage ring in the existing RHIC tunnel to collide with protons and ions. Polarized electrons will be created in the source (shown in red) and accelerated before injection into the electron injector and then into the turquoise electron storage ring. The electrons and the ions could collide at two possible locations of future detectors. The center of mass range for the EIC electron ion collisions will range from 30-140 GeV for electron-proton scattering and up to 100 GeV for electron-ion collisions. The electrons will be polarized, and so will the protons and a few light ions. All possible stable nuclei starting from the proton to Uranium will be provided by the Electron Beam Ionization Source (EBIS).
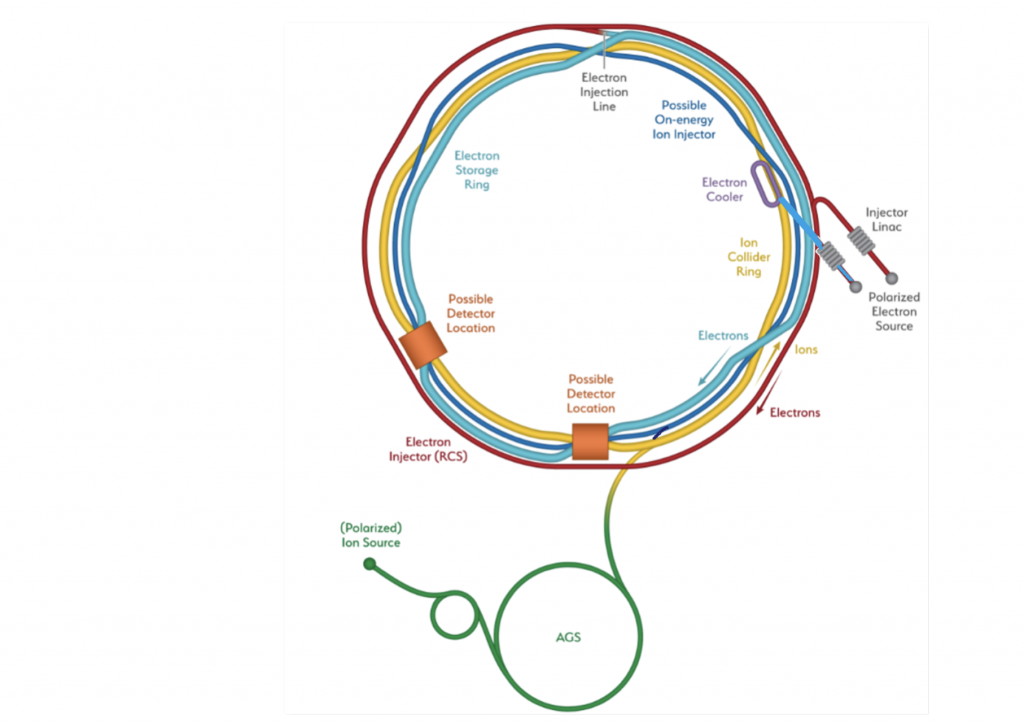
Intensity of the beams in collision results in a quantity called the Luminosity of a collider. Luminosity in units of “# of collisions/unit-area/unit-time” is used to address the number of events the experimenters would see. At the EIC, the desired range of luminosity for its planned physics program is 1033-34 cm-2sec-1. This is 100-1000 times larger than the highest luminosity achieved by an of electron-proton collider ever. These ranges of luminosity and energy of the collider along with the physics goals are summarized in Figure 6. Also shown are two machine performance curves that could be delivered at two interaction regions optimized for different Center of Mass energies.
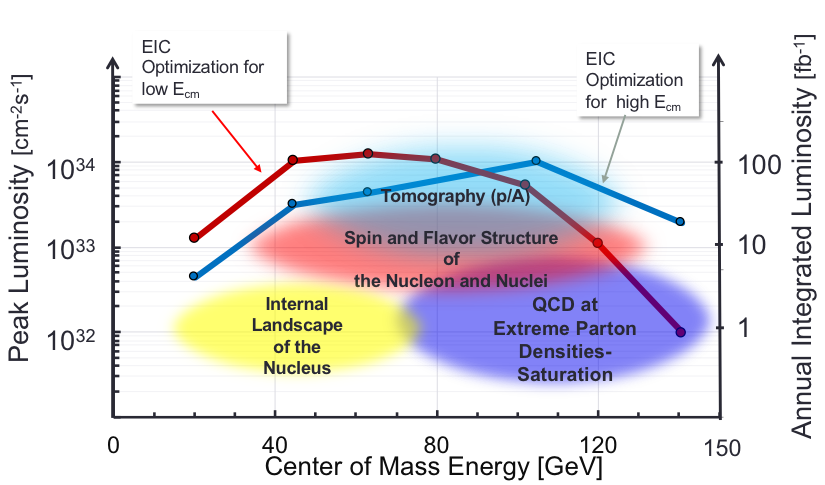
Connections to Other Fields of Physics (selected highlights)
EIC and High Energy Physics
EIC will be a frontier high luminosity facility, with unprecedented potential for achieving high precision. Beyond the Standard Model, enthusiasts are looking into high sensitivity experimental searches for novel physics signals in the EIC’s energy range. The high luminosity of EIC will provide significantly improved flavor separated parton distribution functions (PDFs) and transverse momentum distribution (TMDs) functions. After the Luminosity Upgrade of LHC, the EIC-improved PDFs will be essential for new physics searches at the LHC.
EIC and Lattice QCD
While QCD itself is impossible to solve exactly [5] for various reasons, a discretized version of QCD, in which space and time coordinates become points on a four-dimensional lattice (Lattice-QCD or LQCD) can be solved exactly given enough computing power. Extraction of physics results from LQCD involves limitations due to the finite size of the lattice and the extrapolations to the continuum limit. However, calculations do yield results with possibility of estimating their uncertainties reliably. Recently novel methods have been developed to calculate Quasi-PDFs, and then related them to true PDFs through iterative calculations employing established theoretical tools of Effective Field Theory (EFT) [5]. By the time the EIC will start producing precision data on nucleon spin and related observables, it is anticipated that the novel LQCD tools will also be ready to calculate them with comparable precision, ensuring a new era in QCD studies. LQCD could potentially become the standard tool to interpret the measurements at the EIC and potentially also guide its future program.
EIC and Condensed Matter Physics
At the high energy end of the spectrum of measurements, the EIC will study new types of many-body phenomena, namely the saturation in gluonic matter. At RHIC and LHC, the saturated gluonic state of matter is expected to be present for a short time before it decays into the Quark Gluon Plasma (QGP). It is proposed that the decay of the gluonic matter into the QGP seeds formation of topological defects resulting in the handedness in quark-antiquark pair formation, recently seen in heavy ion collisions, called the “chiral magnetic effect” (CME). Analogues of CME have been seen in condensed matter physics. The initialization of topological defects in heavy ion collisions depends on the details of the color field in saturated gluonic matter whose discovery and study is a central experimental goal of the EIC. With the recent rapid progress in condensed matter physics of novel topological materials suggests possibility of interactions between QCD at the EIC and condensed matter physics to continue in the future.
EIC and Accelerator Physics
Several novel accelerator technologies have been incorporated into the EIC’s design. They serve as intellectual challenges attracting the best of the accelerator scientists to the EIC. Exciting opportunities exist in demonstrating and implementing strong hadron beam cooling necessary to achieve the highest of luminosities. A combination of stochastic cooling and the novel Coherent electron Cooling (CeC) are being developed. Other challenging technologies that will be pushed to their state-of-the-art include superconducting RF, Crab Cavities, those needed to mitigate electron clouds, strong focusing magnets for interaction regions, spin rotators for manipulating beam spin orientation and development of high current polarized sources for electrons and light ions.
A Collider Facility in our Backyard
The physics program at the EIC will be carried out by a worldwide community of nuclear physicists. The EIC user group boasts 1000+ users from 31 countries and is expected to grow by at least a factor of two by the time it operates. The EIC will be the only collider in the US and will operate for ~20 years. With enormously challenging machine parameters it will be a magnet for the brightest of minds in nuclear and accelerator physics research. The proximity of Stony Brook University to BNL is sure to serve both institutions extremely well in attracting scientists interested in the EIC.
It is quite natural that having a major facility in the proximity of a university influences the opportunities available to the faculty, post-docs and students enormously. It is a particular blessing for undergraduate student researchers enabling them to be actively involved in research even while taking classes. Research fields associated with such facilities naturally become strong at the University. The RHIC at BNL impacted the nuclear physics group (one of the top ranked group in nuclear physics the country) over the past two decades. Having the EIC in Stony Brook University’s backyard will ensure this for the next few decades.
The Center for Frontiers in Nuclear Science (CFNS) [6] was established in September 2017 jointly by Stony Brook and BNL, supported by funds from the Simons Foundation and NY State. CFNS has so far focused on supporting EIC science and young scientists involved in the EIC. In a very short time, it established itself as a world-leading Center for EIC science. CFNS currently runs an annual program of international workshops, topical meetings, a visitor program, a post-doctoral fellow program, an annual international summer school and a seminar series. Annually the Center hosts ~300 scientists through such activities. In the EIC era the Center assures an intellectually exciting environment at Stony Brook University. The vision for CFNS is to become a world-leading Center for EIC & broader nuclear science by growing its scope to other frontiers areas in nuclear science, and to the education & support of minorities. •
References
[1] Nuclear Science Advisory Committee (NSAC) Long Range Planning Reports 2002, 2007, 2015; Explicit link: https://science.osti.gov/np/nsac
[2] National Academies of Sciences, Engineering and Medicine. 2018. An Assessment of U.S.-Based Electron Ion Collider Science. Washington DC: The National Academies Press. https://doi.org/10.17226/25171.
[3] Asymptotic Freedom: From Paradox to Paradigm, Frank Wilczek, Nobel Prize Lecture, December 8, 2004; https://www.nobelprize.org/uploads/2018/06/wilczek-lecture.pdf
[4] Electron Ion Collider: the Next QCD Frontier – Understanding the glue that binds us all, A. Accordi et al, Eur. Phus. J. A 52 (2016) 9, 268 Editors: A. Deshpande, Z.-E. Meziani & J.-W. Qiu; arXiv:1212.1701v3 [nucl-ex]
[5] Parton physics on a Euclidean lattice, X. Ji, Phys. Rev. Lett. 110.262002
[6] Center for Frontiers in Nuclear Science (CFNS) http://www.stonybrook.edu/cfns